
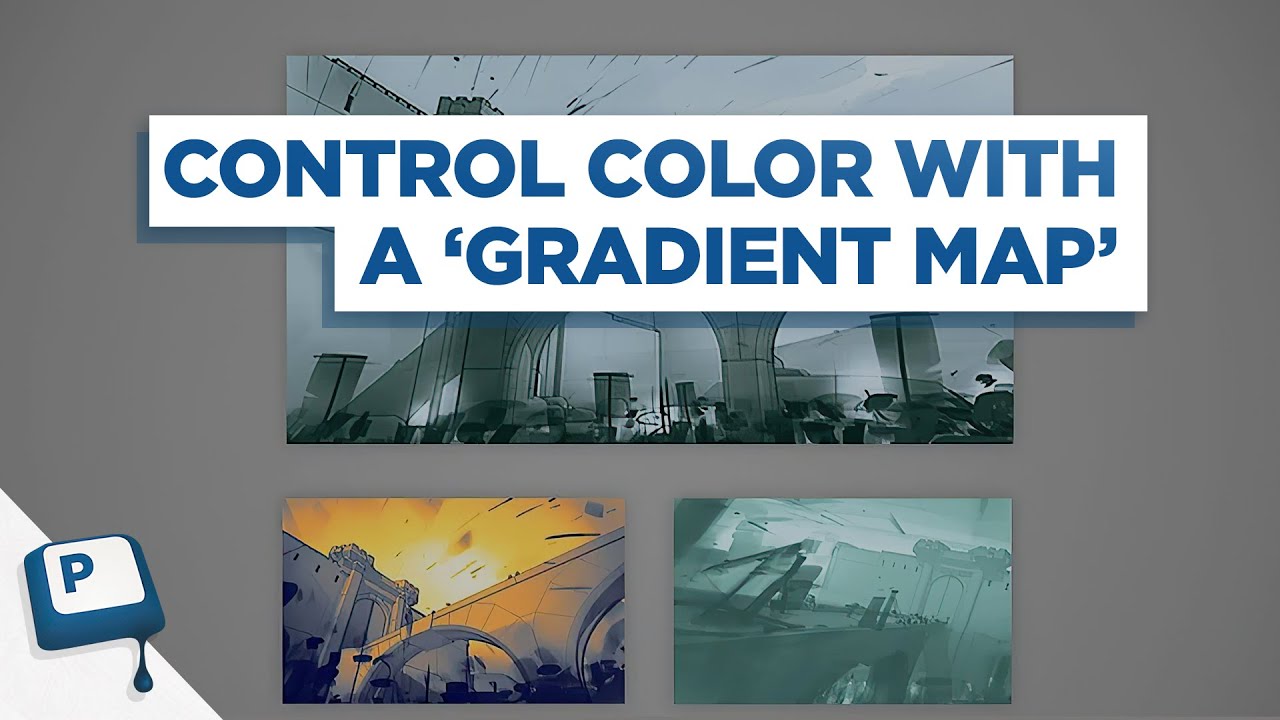
In 1992, Posse ( 21) showed that susceptibility-induced gradients result in an echo-shift in k-space. These two techniques require knowledge about the expected frequency shifts and they are prone to large-scale field inhomogeneities and chemical shift effects. A bright signal in the vicinity of susceptibility can also be achieved using spectrally selective RF pulses either to excite water off-resonance ( 19) or to saturate water on-resonance ( 20). This technique, however, compensates only for susceptibility along the slice direction and requires a priori knowledge about the field disturbance to optimize the slice rephasing gradient strength. The “white marker” technique makes use of susceptibility gradients, i.e., the susceptibility-induced magnetic field gradients along the slice direction that add to a modified slice rephasing gradient, resulting in a high signal intensity in areas with susceptibility and low signal intensity outside these regions ( 17, 18). Therefore, several techniques have been developed that convert the negative contrast due to the local susceptibility into a positive contrast. In particular, tracking of labeled cells can be difficult in tissues with short or heterogeneous distribution of T 2* relaxation times. However, the loss of signal is problematic in many experiments because of poor contrast between the SPIO nanoparticles and the background tissue. In all these applications the local inhomogeneity results in an increased T 2*-decay, and thus a hypointensity in the image. Furthermore, macrophages take up USPIOs by phagocytosis, which can be used to study atherosclerotic plaques and other inflammatory processes ( 14 – 16). Labeling cells (e.g., stem cells) with SPIO nanoparticles allows for the monitoring of the temporal and spatial migration of the cells into target tissues ( 11– 13).
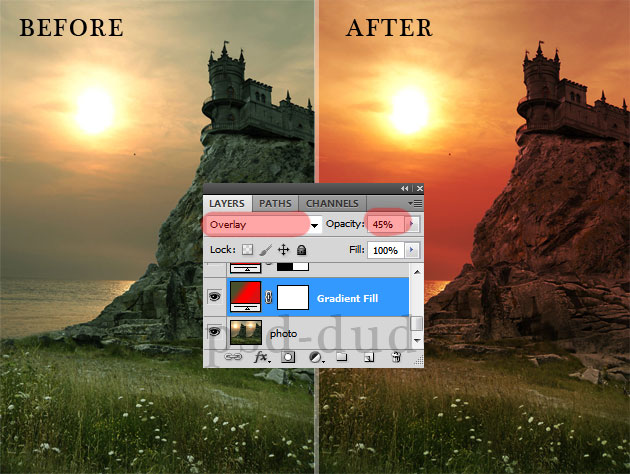
Similarly, ultrasmall SPIO particles (USPIOs) are used as blood pool agents in MR angiography ( 10). Especially, super-paramagnetic iron oxide particles (SPIOs) are currently used for liver-specific MRI ( 7) or the detection and characterization of lymph nodes ( 8, 9). This T 2* decay is exploited as the source of image contrast in various applications, such as functional MRI ( 1, 2), passive tracking of interventional devices ( 3, 4), the visualization of iron deposition in tissue ( 5, 6), and the measurement of susceptibility-inducing contrast agents.

Any local inhomogeneity in the static magnetic field results in the dephasing of the precessing magnetic moments, and thus in a fast decay of the NMR signals. Usually, a homogeneity in the range of parts per million (ppm) is required to measure an NMR signal. MRI is based upon the use of a homogeneous static magnetic field for the detection of coherently precessing magnetic moments. The method bears the potential also for usage in other applications, including the detection of contrast agents and interventional devices as well as metal implants. In vivo experiments showed the feasibility of the method for tracking of SPIO-labeled cells. Phantom experiments demonstrated that local susceptibility differences can be quantified. The susceptibility gradient map is determined in a postprocessing step, requiring no dedicated pulse sequences or adaptation of the sequence before and during image acquisition. The proposed method calculates the susceptibility gradient and visualizes it in a parametric map directly from a regular gradient-echo image dataset. In this work, a new method is proposed to selectively turn this negative contrast into a positive contrast. In particular, cells labeled with superparamagnetic iron oxide particles (SPIOs) induce hypointensities, making the in vivo detection of labeled cells from such a negative image contrast difficult.

Local susceptibility gradients result in a dephasing of the precessing magnetic moments and thus in a fast decay of the NMR signals.
